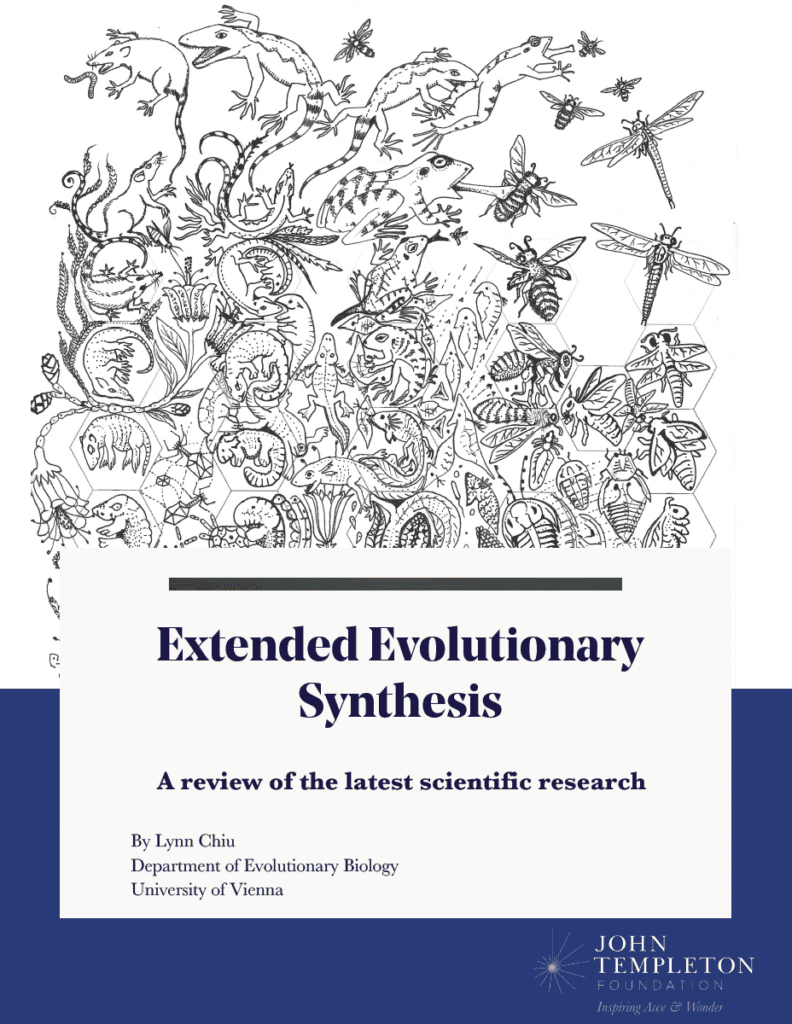
Cover page of a report on Extended Evolutionary Synthesis.
This review—intended for curious readers, reporters, researchers, and teachers—is a road map to navigate rapidly moving terrain. To understand the direction of emerging research today, we need to ask: What happened to evolutionary biology during the 20th century and what did it leave out? Why is the Extended Evolutionary Synthesis seeking a more inclusive approach to evolutionary theorizing? And finally, what does a comprehensive evolutionary theory look like?
The review proceeds in three parts: examining the genetic turn of evolutionary theory since Darwin (Part 1); sampling multiple, independent calls for an alternative outlook (Part 2); and finally, taking a close look at the emerging structure and outcomes of an Extended Evolutionary Synthesis research program (Part 3).
Read on for excerpts from Part 1 and 2 of the review, and read the full review here.
How to Cite:
Chiu, L. 2022. Extended Evolutionary Synthesis. A Review of the Latest Scientific Research. John Templeton Foundation. West Conshohocken, Pennsylvania, USA. 85 pp. 10.15868/socialsector.40950
Extended Evolutionary Synthesis: Part I
How Evolutionary Theory Became a Gene-Centric Theory of Evolution
Students taking their first course in evolutionary biology will often find that evolution is not what they expected. To address questions like “why do dinosaurs have feathers” or “how does this parasite come to have a life cycle through ants, birds, and cows,” they will learn that they need to look past the complex features and zoom in on where the evolutionary action actually lies: at the level of genes. Instead of studying how these traits develop and function, the students will find themselves busily calculating the relative numbers of gene variants and squinting over alignments of genetic sequences.
Evolutionary theory—as it is practiced today—is about changes in the genetic makeup of populations. It’s about random genetic mutations and their recombinations, about the long reach of genes into the selective environment, and about the faithful transmission of genetic information across generations. To explain the evolution of phenotypes is to explain the evolution of their underlying genes.
The standard curriculum of evolutionary biology is a product of nearly a century of intense focus on what the genetic world can offer to the rest of biology. The backbone of this curriculum is the Modern Synthesis, a theoretical discipline that has dominated evolutionary biology since its maturation in the mid-20th century. Instead of investigating evolutionary questions in terms of phenotypes, morphologies, embryonic structures, or ecological relations, the theories, practices, and training programs under the Modern Synthesis focus solely on the micro-evolution of genes.
However, the evolution of small genetic variants is merely one aspect of the complex evolution of life. For decades, scholars have expressed their deep frustrations over the limitations of the Modern Synthesis approach. They proposed amendments, revisions, expansions, or replacements that can enable us to return to the study of phenotypes and organisms, and how they interact with their environments. Some return to early Darwinian or even pre-Darwinian thought to revive discarded ideas. Others embrace theoretical insights from other disciplines, such as physics and complexity science.
This research review takes a tour through five major episodes of the last 150-plus years to understand how evolutionary biology became a gene-centric theory of evolution. One aim of this short survey is to help us better understand the specific concerns that motivated an “extended evolutionary synthesis (EES),” which started to gain substantial ground in the early 2000s. A common misconception is that the EES is a full-blown refutation of Darwinian evolutionary theory. It is not. Understanding the history of evolutionary biology will show us that it has never been a single, static, or unified research field, but a dynamic constellation of concepts, assumptions, and practices. Not everyone agrees with the entire package, but no respectful scientist would reject them all.
Let us begin by thinking through what people mean by the term “Darwinism.”
Darwin and Darwinism: Mid-19th Century
Darwin’s contribution to biology and our worldview was revolutionary (Mayr 1993; Ayala 2007). He collected compelling evidence that all life on Earth descended from a common ancestor, forming a single “Tree of Life.” Darwin’s principle of common descent (or descent with modification) states that species are not individually created but instead come into being by branching off from each other. Species are not fixed, essential categories, but populations of individuals with a variety of minute differences.
Darwin also proposed the theory of natural selection, where natural selection is the natural consequence of an exponentially growing population pushing up against finite, linearly growing resources. The result is a “survival of the fittest,” with the supposedly “fitter” (better adapted) types surviving better and reproducing more, and able to pass down their fitter traits to the next generation(s). Gradually, evolution by natural selection results in the accumulation of small differences into complex adaptations.
We can think of the evolutionary tree as a map that represents how species are connected to each other. Evolution by natural selection is the engine that forged these connections by gradually splitting a population into new branches and pushing them further into greater differences.
Even though Darwin’s main contribution to evolutionary biology was the theory of evolution by natural selection, he also accepted other modes of evolution, for instance, the principle of use and disuse often attributed to Jean-Baptiste de Lamarck (1744—1829) (i.e., traits strengthened through constant use in the parental generation are inherited by the offspring as a stronger trait, whereas traits that are not frequently used are only weakly inherited).
Furthermore, it was not clear to Darwin how inheritance works. He considered a variety of ways organisms may have been able to “like beget like.” In addition to the inheritance of factors that are transmitted without influence from the life experiences of parents, he also considered various ways parental life events can “leak” into future generations through the inheritance of acquired characteristics, also frequently associated with Lamarck. Darwin’s own theory was thus partially “Lamarckian.”
Nowadays, the term Darwinism is often associated with the following assumptions concerning the evolution of natural selection:
Gradualism is the idea that populations could generate an abundance of variations, each minutely different from each other. As mutations are small, evolution works at a slow, leisurely pace, gradually sculpting and building up traits one tiny step at a time until they accumulate into complex forms.
Some argued that for natural selection to work, the individual traits of an organism should, to a large extent, evolve relatively independently of each other. From this atomistic perspective, natural selection can accumulate complex forms because it can safely tinker with one part of the organism without also changing the other parts.
Finally, the late Richard Lewontin (1929—2021) noted that Darwin’s theory cut a sharp line between organisms and environments. Many predecessors and contemporaries of Darwin did not differentiate between the influences from organism and environment in evolution—individual changes feed into and are part of evolutionary change. Darwin, however, split the world into the organismal and environmental domains, with natural selection shaping the organisms to match up with a pre-existing environment.
As Lewontin put it, natural selection is the process of organisms proposing new traits that the environment disposes. Organisms blindly throw up variations that may fail or succeed while the environment determines the winners and losers. New variants do not emerge predetermined to meet preexisting environmental challenges, but are instead randomly generated and put to the test. As a result, the direction of evolution is entirely imposed by the external environment (externalism) (Levins and Lewontin 1985; Godfrey-Smith 1996). In sum, a Darwinist is mainly committed to the following theses: evolution as a Tree of Life, the theory of natural selection, gradualism, atomism, and externalism.
Extended Evolutionary Synthesis: Part II
How Development Drives Evolution
During the development of multicellular organisms, a single cell divides and proliferates into an aggregate of cells, which then acts as a coherent entity. It then undergoes reshaping into the specialized tissues of a developing embryo. A common misunderstanding of this process treats development as a mere readout of an evolving “genetic blueprint.” Development—a proximate cause—is thus seen as irrelevant to evolution. Indeed, the fields of embryology and developmental biology were not included in the construction of the Modern Synthesis and were left out of evolutionary biology (Gilbert et al. 1996).
The field of evolutionary developmental biology (“evo-devo”) is one of the major contributors to a new theoretical framework of evolutionary biology (for instance, Amundson 2005; Müller 2007, 2020; Carroll 2008; Moczek 2012). Under evo-devo, evolution is not treated as heritable changes in gene frequencies, but as the evolution of the phenotype through heritable changes in development.
Increasingly, evo-devo studies can be divided into two sets of questions (Figure 6): “traditional” evo-devo asks how development evolves. A “devo-evo” (developmental evolutionary biology, or developmental evolution) branch of evo-devo, on the other hand, examines the direct impact of developmental processes on the strength, rate, direction, and dynamics of evolution (Gilbert 2003a; Müller 2007; Moczek 2012). Devo-evo studies flip our understanding of the relationship between development and evolution. Instead of thinking of developing organisms as a passive putty molded and sculpted by natural selection, development is instead found to be a complex and dynamic process actively guiding phenotypic evolution.
Consider the problem of “hot spots” of evolutionary innovation and diversity in regions that are normally conserved across species. Beetles exhibit a fantastic array of big, small, and oddly shaped horns on the top of their heads (i.e., thoracic horns at the first thoracic segment). These thoracic horns are novel traits in evolution, while the head itself is deeply conserved across species.
Armin Moczek and his team found that the horns, as true novel traits, did not evolve from the appearance of new genes alone. Instead, these evolutionary innovations also repurposed the developmental circuits of other structures (Hu et al. 2019a, b; Linz and Moczek 2020). One of these is the so-called “pupal horns” of beetle pupae that help them break through head capsule cuticles. A surprising finding is that evolutionary pathways used for the development of wings were also co-opted for the development of adult horns!
One take-home message from these studies is that we need to reexamine the orthodox idea that there are such things as “gene-for X” (e.g., “genes for wings” or “genes for horns”). As the genetic networks of early developmental processes were used in later or other contexts, basic developmental packages could become involved in multiple phenotypes and their evolution. New traits are not always caused by the appearance of new genes.
A more general lesson is that developmental processes can influence which phenotypes can actually appear, how they appear, how likely, and how frequently (Uller et al. 2018). Many argue that development can thus “bias” the direction of evolution. Minimally, when certain traits cannot develop, they cannot evolve. Developmental processes can further dictate how organisms evolve as well. The study of “developmental bias” is a core component of evo-devo/devo-evo studies (Alberch 1980; Maynard-Smith et al. 1985).
To visualize developmental bias, we can make use of what is known as a “morphospace” or phenotypic space. Let us image evolution as carving out a path in a multidimensional space of physically possible traits. Each axis in the morphospace represents variations on a phenotype. Each dot stands for a possible organism with a combination of traits. Standard evolutionary theory tells us that genetic mutations are small and abundant as well as randomly generated. This will generate a densely covered, evenly populated morphospace (Figure 7A). Natural selection can connect the dots to draw out any path within this space (Figure 7B).
The study of developmental bias has revealed that natural selection is not the only game in town. The process of development—how organisms change throughout their life cycles—can have a major impact on the direction, rate, and magnitude of evolution. In some cases, developmental features can prevent certain types of traits from developing, even if there is an abundance of small genetic mutations. In other cases, there is a “canalization” of phenotypes, that is, even as genetic mutations accumulate, there is no new phenotypic expression as phenotypes are stabilized by multiple physiological and developmental processes. Using the two-dimensional morphospace as a metaphor, we can see that the morphospace is not fully filled (Figure 7C). Evolution can only push in a few directions because there are “developmental constraints” against the other possibilities (Gerber 2014).
Yet the impact of developmental architecture on evolution is not merely “negative” (in that it restricts evolutionary possibilities). From the perspective of standard evolutionary theory, natural selection can still act as it pleases as long as the available phenotypes are still the result of an abundance of small, genetic mutations that map onto phenotypic variation.
A key characteristic of developmental bias is that changes to developmental processes can also create novel variants in response to genetic or environmental perturbations. Developmental processes are complex dynamics that involve multilevel interactions, from the genetic level to the tissue and organismal level. These dynamics also include inputs from the environment.
Changes at any of these levels, not just genetic mutations, can trigger coordinated responses across multiple levels, thus generating novel phenotypes. This type of phenomenon is sometimes called “facilitated variation” (Kirschner and Gerhart 2006; Gerhart and Kirschner 2007) or “emergent variation” (Badyaev 2011).
Understanding bias as not just the constraining (developmental constraint) but also the constructive effects (facilitated variation) of development on evolution allows us to think about the complex ways development can reciprocally interact with natural selection (Félix 2012; Uller et al. 2018; Salazar-Ciudad 2021). Against gradualism, the reality may be that while simple traits may evolve through gradual accumulation of small variants, complex traits will tend to evolve through quick leaps (“punctuation”) (Salazar-Ciudad and Jernvall 2005).
Biological structures with serial repeated characters such as the teeth, fingers, insect segments, or pigmented patterns are classic model systems for the study of developmental constraints. These repeated units look alike because they share similar or the same developmental “modules.” They are thus excellent systems to examine how tightly integrated these developmental modules are (“modularity”), which can constrain possibilities in a morphospace. The properties of these modules can determine how easy or difficult it is to evolve new variations (“evolvability”).
For instance, analyzing the global genetic database of Maine Coon cats, which have a tendency toward polydactyly (the possession of extra digits), Lange et al. (2014) found that these extra digits are not generated randomly. Physicodevelopmental processes result in strong tendencies to develop repeats on only specific digits.
Another example of developmental constraint is the eyespots of butterfly wings, which are often repeated along each wing and on both sides. They look stunningly like the eyes of snakes or owls, confusing predators. In the wild, the two main eyespots of African Mycalesina butterflies naturally vary in their size as well as the relative colors of their concentric rings (some have thicker black bands than others).
Patrícia Beldade, Paul Brakefield, and others have examined how far they can push the independent evolution of the two eyespots by artificially selecting for different eyespot colors and size (Brakefield et al. 1996; Beldade et al.2002). Standard evolutionary theory would predict that we could push the populations to evolve in any direction (that is, any combination of eyespot size and color) as there already are natural variations of these traits in the population to select from.
Yet after evolving the species Bicyclus anynana for multiple generations, they found that only the size of these eyespots fit this prediction. It is relatively easy to evolve the sizes of each eyespot independently (they could easily evolve butterflies with one big and one small eyespot). However, it is not quite possible to do so with the colors (they couldn’t easily evolve one eyespot with a big black band while the other has a thinner black ring) (Allen et al. 2008). The difference between size and color is explained by their respective developmental structures, which shows that preexisting genetic variation is not enough for selection to create new types. The developmental constraints must allow it.
Interestingly, in one species, artificial selection can freely evolve butterflies with eyespots that vary in color composition (Brattström et al. 2020). The appearance of these new types enabled a burst in diversity of new eyespot morphologies in that lineage. This shows that when evolution breaks through developmental constraints, the previously impossible variations are now available for selection.
Why Physics Matters for Evolution
The gene-centric focus of standard evolutionary theory can easily distract us from the effects of physics on the developing organism.
A striking example is the role of gravity in chick development (Kochav and Eyal-Giladi 1971). Eggs rotate and spin at a regular speed as they pass through the hen’s reproductive tract. While rotating, the lighter elements remain at the top end of the yolk thanks to the presence of gravity. This region is important—it marks the back end of the future chick. Gravity and its effects on egg development are clearly not part of the genetic program but are essential for the shaping of the embryo.
Two crucial facts about the developmental process are commonly ignored under the genocentric framework: (1) that it is the physical properties of cells that determine how they move, stick together, stop, or separate from each other and (2) that the genes and proteins of a developing embryo often act by harnessing the resultant mechanical forces and fluid flow in the specification of organismal form. In the molecular era of the 20th century, these physical and mechanical aspects of development have been sidelined in favor of explanations focused exclusively on molecular signaling pathways and genetic circuits.
Stuart Newman has long championed that biology should take seriously the importance physical factors such as adhesion, surface tension, viscosity, phase separation, gravitational effects, etc., that determine organismal form (Newman and Frisch 1979; Newman and Comper 1990; Newman 1994). As these physical properties are also characteristic of some kinds of nonliving matter, they are “generic” physical mechanisms that inherently generate shapes and forms, for instance, the formation of cavities, layers, segments, tubes, and appendages. The origin of complex multicellular form appeared before the evolution of complex genetic programs. It was physical processes, with early genes enabling cells to access the generic physical mechanisms, that made multicellularity possible.
An example is cell adhesion. Cells stick together in multicellular organisms through adhesive proteins on their surfaces. In animals, cadherins typically perform this role. Even though these proteins are the products of genes, they don’t acquire their function—stickiness—without the right environment. Cadherins depend on calcium for their function; they are not sticky in the absence of this ion. A sudden shift in environmental calcium can thus transform single cells into multicellular masses, a major morphological transition based in biophysics. Other examples include surface tensions and electrophysiology (Foty et al. 1996; Brodsky and Levin 2018).
The major body forms we see today were created early in the evolutionary history of animal life (Newman 2016). From the physico-genetic, “biogeneric” perspective, the new forms did not evolve through the long accumulation of small genetic mutations. Instead, the major morphological motifs or features can be explained as the natural result of their unique physical properties and mechanisms (Newman 2002; Newman et al. 2006).
For instance, separation between cell groups arises when there are differential adhesions of cells. Cavities and lumens form when there is asymmetric distribution of adhesion molecules on cell surfaces. Patterns that can shape future tissues come from sedimentation by gravity, or the diffusion of morphogens and their interaction with the cells’ gene expression. An ancient multicellular animal might have been able to easily shift between these different forms depending on the balance of these factors. As evolution proceeds, however, some morphologies could become locked into a stable state when selection favors genetic changes that further stabilize these states.
When considering convergent traits between species, rather than attributing their similarities to similar sources of natural selection, it is also important to consider whether their traits arise from shared generic physical mechanisms. In their latest paper, Arias et al. (2020) argue that the similar developmental trajectories of myxobacteria and dictyostelids are more due to shared generic physical processes and similar agent-type behaviors.
Standard evolutionary theory assumes that new, complex, and adaptive forms evolve only through the accumulation by natural selection of random, small changes in genes. By studying the roles of physical effects in morphogenesis, physico-genetic arguments show that phyletic transformations can be based on transitions in bio-physico-chemical properties and mechanisms inherent to the developing animals (Newman 2019). The genetic underpinnings of these transformations can be novel toolkit genes that endow the developing tissues with new inherent morphogenetic propensities (the principle of inherency). But they could also be existing genes for which environmental effects elicit new physical properties, with the morphological outcomes reinforced by subsequent evolution of stabilizing genetic interactions. These studies show that we need to refocus on the physical mechanisms and properties that matter, not just genetic information.
Still Curious?
Read the full research review on Extended Evolutionary Synthesis by Lynn Chiu
Explore our other research reviews on topics such as: